The Evolution Of Gene Therapy: From Small Market Diseases To Broad Patient Populations
By David Kirn, M.D., Co-founder & CEO, 4D Molecular Therapeutics
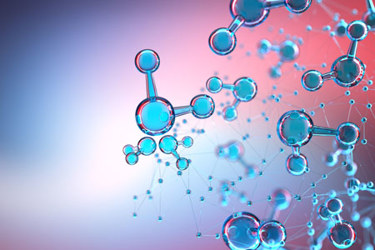
Gene therapy and other genetic medicines hold great promise for the treatment of patients with high unmet medical needs. However, to date, gene therapy successes have been limited to rare, monogenic recessive diseases. With innovation and vision, AAV-based genetic medicines have the potential to treat large market, complex diseases that affect hundreds of thousands of patients every year. To achieve this vision, our industry must learn lessons from the development of past and current AAV-based gene therapies. These lessons can be used to direct cutting-edge innovation to overcome hurdles with current vector systems. This gives us, as an industry, the opportunity to unlock the full potential of AAV vector-based genetic medicines for countless patients with complex diseases.
The Emergence of Gene Therapy as a Treatment Modality: Promise Followed by Disappointment
The original application of traditional gene therapy several decades ago was focused on rare monogenic diseases. Examples included the treatment of patients with severe combined immunodeficiency syndrome (SCID) with retrovirus-based therapeutics, as well as monogenic liver disorders (e.g. ornithine transcarbamylase deficiency, or OTC deficiency) with adenovirus vectors. While promising clinical activity was demonstrated in each case, these first-generation vectors resulted in significant toxicities. In the case of retrovirus-based therapeutics for hematologic diseases such as SCID, gene therapy treatment ex vivo led to leucocyte proliferative disorders and even leukemias in some cases. Adenovirus vector toxicities were generally related to high levels of inflammation due to the virus itself, including severe hepatotoxicity with intravenous administration. In addition to these limitations of the vector systems used, another challenge during this era was the application to complex large market diseases such as cancer; these diseases were unlikely to be cured with delivery of a single transgene product.
These pioneering but ill-fated programs advanced the field by demonstrating the potential of gene replacement therapy. However, these products also made it clear that safer and less inflammatory vectors were needed. These results set the stage for the emergence of AAV vectors as the preferred delivery vehicles for in vivo treatment of non-proliferative tissues.
AAV Gene Therapy 1.0
The discovery that the capsid of the adeno-associated virus (AAV) could be used as a vector to deliver a transgene cassette revolutionized in vivo gene therapy. Lentivirus vectors had the same impact on ex vivo gene therapy and cell therapy with hematopoietic stem cells; these viruses were able to insert safely into the genome (without causing leukemias) of target proliferative cells and therefore sustain transgene expression despite cellular dividing and proliferation. For in vivo gene therapy, AAV vectors were found to be less immunogenic than
adenovirus, and they did not have the high risk of insertional mutagenesis associated with retroviruses. With a carrying capacity of approximately 4.7 KB, these vectors had the potential to deliver a therapeutic transgene together with a promoter sequence to drive high-level transgene expression.
However, the lack of insertion into the genome limited these treatments to non-proliferative tissues such as the retina, other neurologic tissue (e.g. brain, spinal cord, or dorsal root ganglia) and to a lesser extent the liver. Hematologic or gastrointestinal diseases were therefore not treatable with these AAV vectors; tissue targets were therefore limited to stable and relatively non-proliferative tissues. In addition, the capsid size constraints limited delivery of transgenes based on size; delivery of large genes such as microdystrophin or full-length cystic fibrosis transmembrane regulator (CFTR) were not feasible. For large transgenes that did not fit into AAV vectors, transgene innovations were used to design and construct truncated but functional protein sequences that fit into the AAV capsid. Finally, the naturally occurring wildtype AAV capsids available were inefficient at target cell transduction in many cases, and they were not targeted for delivery to target tissues by routine routes of administration.
The initial successful AAV-based gene therapy products were therefore designed and developed for monogenic recessive diseases, with transgenes that fit into AAV (i.e. --
While these early proof of concept AAV-based products had profound impacts on patient outcomes in some cases, these products also demonstrated the limitations with first generation AAV gene therapy products and vectors. Given the difficult and inefficient routes of administration for extrahepatic tissues such as the retina or skeletal muscle, these conventional AAV vectors are not feasible delivery vehicles for gene therapy products addressing large market diseases that impact hundreds of thousands of patients per year. In addition, the inefficient delivery and transduction with these vectors has led to relatively high doses with high cost of goods that are not acceptable for large market diseases.
AAV Gene Therapy 2.0: AAV-based Genetic Medicines
Despite the success of these products for niche, rare monogenic diseases, the need for better AAV vectors has become increasingly clear over time. The field needs vectors that are more efficient at transducing specific cell types, and vectors that are more efficiently delivered at low and safe doses by routine routes of administration clinically (e.g. intravenous, aerosol, or intravitreal). In addition, pre-existing anti-AAV antibodies has led to the exclusion of up to 50% of patients on clinical trials as well. Vectors that do not cross react with pre-existing antibodies in the population are therefore an attractive feature of any new target vector profile. With targeted vectors delivered by routine routes of administration, the safety and immunogenicity of these products should be superior to first generation products. Cost-of-goods (COGs) should also be more attractive, especially for large market diseases.
Two general approaches have been identified for the invention of superior AAV vectors. First, rational design approaches were utilized to identify vectors with superior trafficking to the nucleus of target cells (e.g. tyrosine mutants). In addition, GH loop modifications have been made to reduce binding and inhibition by pre-existing antibodies in the population. However, the limitation of these rational design features is that they each address a single step in a very complex and multi-step process from a vector leaving a physician’s needle and getting all the way to the nucleus of target cells within a particular tissue.
In contrast to rational design, directed evolution has the potential to correct and optimize multiple steps in the journey of a therapeutic capsid from needle to nucleus. Briefly, directed evolution is a Nobel Prize-winning technology that allows humans to leverage the power of evolution to create novel bespoke biologics. In the case of AAV, the capsid can be diversified in order to generate billions of potential synthetic AAV capsids. In vivo iterative selection can then be used to identify the synthetic AAV capsid with the best match to the investigator’s target vector profile. These selections are best done in non-human primates in order to increase the likelihood of translation into humans. In addition, in order to increase the likelihood of success in humans, selections can be performed in parallel or sequentially on human cells and/or human organotypic tissues. Finally, through exposure of the vector library to pools of antibodies from the human population (e.g. human IV-Ig), investigators can identify vectors that meet the target vector profile and simultaneously, are relatively resistant to pre-existing antibodies in the human population. Therefore, more patients can be enrolled and treated on clinical trials and after marketing approval.
Directed evolution has been utilized to invent customized capsid vectors for a variety of tissues. These include intravitreal retinotropic vectors for delivery to the retina, aerosol delivered vectors for the lung airways, and low dose intravenous vectors targeting extrahepatic tissues such as the heart. While products utilizing these novel, customized and evolved vectors have yet to be approved for general use, they hold the potential to enable treatment of large market disease indications where safety, COGs, and routine administration are paramount.
Future Directions Beyond AAV Vectors: Non-Viral Nanoparticles and Oncolytic Viruses
The future of AAV-based genetic medicines is bright. Transformative gene therapy medicines for rare monogenic recessive diseases have been approved, including Zolgensma (for spinal muscular atrophy, or SMA), Luxturna (for Leber congenital amaurosis type 2, or LCA2, and other conditions due to RPE gene mutation) and Hemgenix (for hemophilia B). In addition, next generation AAV-based genetic medicines are being developed for large market diseases such as neovascular age-related macular degeneration (wet AMD), diabetic macular edema, inflammation, and cancer.
Nevertheless, hurdles still exist for next generation optimized AAV-based medicines. First, while AAV capsids can be invented that do not cross react with pre-existing immunity, and these can be combined with sophisticated immunosuppressive regimens, anti-AAV capsid immunity will nevertheless arise in most patients following treatment; repeat dosing may therefore be challenging for tissues that turn over and lose gene expression over time. Second, the transgene carrying capacity of AAV is limited by its physical shape and size. Larger transgene cassettes, such as are required for full length microdystrophin or most CRISPR/Cas-based gene editing constructs, are therefore currently not deliverable with AAV vectors. Finally, since AAV does not genetically modify nor integrate into the DNA of the target cell, AAV-based genetic medicines will not lead to durable gene expression in highly proliferative tissues such as the gastrointestinal tract or hematopoietic stem cells.
Next generation delivery systems for genetic medicines are therefore needed. Non-viral transgene delivery systems include polymers, lipids (e.g. liposomes), peptides, and hybrid lipid nanoparticles (LNP). Approved genetic medicines utilizing LNP delivery vehicles to the liver include Onpattro (patisiran, siRNA inhibiting transthyretin translation for ATTR) and Leqvio (inclisiran, siRNA inhibiting PCSK9 translation for heterozygous familial hypercholesterolemia). Pegylated LNP vectors for RNA-based COVID-19 vaccines were hugely successful and transformative for society.
Non-viral delivery systems have the advantage of being less immunogenic, allowing for the potential of redosing. In addition, non-viral gene delivery systems may have larger transgene payload carrying capacities. With larger payload carrying capacities, gene editing within diverse and large market patient populations may become increasingly feasible over time. These non-viral delivery vehicles can also be “decorated” by PEG or targeting proteins such as antibodies.
The downside of these systems remains the inefficient transduction of cells overall, with highly inefficient trafficking to the nucleus (as is required for DNA based therapeutics). In addition, delivery and transduction with these systems is not targeted generally, except for liver-directed particles. Gene delivery systems for targeting extrahepatic tissue are needed for the treatment of most large market diseases.
Oncology applications require differentiated delivery systems. Given the need to treat systemic, metastatic cancer deposits, and because cancer cells proliferate and mutate over time, standard replication deficient AAV or non-viral vector systems are unlikely to be successful. Replication-selective oncolytic virus vectors are an attractive alternative for the treatment of metastatic cancers. These viral vectors are engineered to replicate selectively within cancer cells, while their replication and gene expression is blocked in normal cells. Several different strategies have been utilized to generate tumor selectivity. First, gene deletion mutants have been engineered for cancer selectivity by deleting viral genes that are necessary for replication in normal cells, whereas these same viral genes are expendable for replication in tumor cells. Examples include deletion of the thymidine kinase gene in vaccinia virus or herpes virus, and deletion of E1A gene regions (e.g. conserved region 2) or the E1B-55k gene in adenovirus. Another approach is to use cancer-specific promoters to drive critical genes for viral replication in cancer cells selectively.
To maximize efficacy for metastatic cancers, beyond what results from viral replication and associated cancer cell death alone, these oncolytic viral vectors are commonly engineered to express additional anti-cancer gene products. These “armed” oncolytic viruses can be engineered to express various immunostimulatory transgene products to generate an “off the shelf” patient-specific cancer vaccine; these agents hold great promise in combination with immune checkpoint inhibitor antibody therapies (e.g. PD-1 inhibitors). These agents can also express transgenes to enhance tumor cell sensitivity to chemotherapy and/or radiotherapy. Armed oncolytic viruses hold great potential for the multi-mechanistic treatment of patients with metastatic cancers.
In summary, the future of genetic medicine and gene therapy is extremely bright. Innovations in the design and engineering of vectors, transgenes, and promoter elements are revolutionizing the field. Customized immunomodulatory regimens are also enhancing the safety, efficacy, and re-dosing feasibility of gene therapy. These innovations will expand the potential of gene therapy to hundreds of thousands of patients with large market, complex diseases.
About the Author
David Kirn, M.D., is co-founder and CEO of 4D Molecular Therapeutics (4DMT), a clinical-stage biopharma company inventing and developing innovative products to unlock the full potential of genetic medicine to treat large market diseases. Dr. Kirn is also Adjunct Professor of Bioengineering and Molecular & Cellular Biology at UC Berkeley.
Over his 30-year career, Dr. Kirn has co-founded and been CEO of four viral vector-based genetic medicines companies, three of which to date were either acquired or went public. Dr. Kirn also held senior clinical research and development positions at Onyx Pharmaceuticals (VP) and Celgene (SVP), and was a senior advisor to Novartis, Bayer, Pfizer, Biogen and others in the genetic medicines field.
Dr. Kirn received a BA in Physiology (Departmental Citation; Phi Beta Kappa) from UC Berkeley in 1985, an MD (Alpha Omega Alpha) from UC San Francisco Medical School in 1989 and completed internal medicine residency training at Harvard Medical School, Brigham and Women’s Hospital (including a term as Chief Medical Resident at affiliated VA hospital). He has also completed hematology-oncology and clinical research fellowships at UC San Francisco and completed a certificate of business excellence from the Haas Business School at UC Berkeley. In 2013, he was awarded the Johnson & Johnson Entrepreneur Innovator award from the J&J Innovation Center.