In Vivo Genetic Medicines Need a Guide: The Answer Lies in Harnessing the Transcriptome
By Sophia Lugo and K. Eerik Kaseniit, Co-Founders, Radar Therapeutics
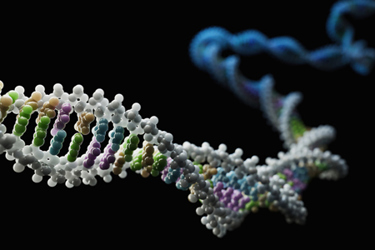
The DNA/RNA Revolution is Underway. Solving Specific Targeting is Next.
Medicines programming, rewriting, or inserting DNA or RNA promise faster therapeutic development, versatile application to a wide variety of diseases, and personalized disease-modifying solutions. However, both DNA- and RNA-based genetic medicines face the same essential challenge: they generally lack specificity for target cells in vivo. In vivo modifications in the wrong tissue or cell type can cause toxicity by disrupting the function of healthy cells and increasing the chances of dangerous immune reactions. Precise targeting mechanisms are needed for safe and effective in vivo genetic medicines, and to bring the promise of genetic medicines to a wider range of patients.
mRNA delivery: Targeting strategies for LNPs
mRNA Modulation Through miRNAs, Codon Optimization
In addition to vector engineering, the sequence of the mRNA payload itself can be modified to target its expression. For example, microRNA (miRNA) target sites can be incorporated into the payload as “off switches,” enabling degradation or silencing of the mRNA in cell types that highly express the cognate miRNA. While this can enable an “off-switch” that avoids expression in a specific cell type, miRNA-enabled “on-switches” that only express in a target cell population and nowhere else require significant further engineering and complexity that are likely infeasible in humans. In the “off-switch” scenario, since the mRNA defaults to an “on” expressing state, complexities can arise around dynamics. For example, mRNAs may start making the protein before the target miRNAs have time to turn “off” translation. Dosing is also an issue, as the non-target cell must express enough miRNA to silence all therapeutic mRNAs delivered to them without having off-target effects from depleting the available miRNA pool from their endogenous targets. Furthermore, miRNAs constitute only a small percentage of genes (around 5%) and are rarely the primary drivers of disease states, requiring significant experimental effort to validate their effects on transgene expression and limiting their utility to certain cell types.5,6 No therapeutics involving synthetic miRNA binding sites have made it to the clinic, even though the concept has been described for a long time.7
In another approach, it has been noted that certain cancers with mutations in TP53 are known to exhibit higher rates of stop codon readthrough.8,9 This property has been used to engineer mRNAs with higher expression levels in certain cancer cells over healthy cells.10 However, it is unclear whether differences in readthrough rates are sufficient, particularly as many regulatory factors are thought to affect stop codon readthrough. Regardless, this strategy is not broadly generalizable to other cell types or states.
Obstacles with Current Delivery Technologies
Like for mRNA, technologies for gene delivery have their own set of challenges that limit their effectiveness and safety. Here we will discuss two broadly used vectors (AAVs and lentiviruses) as well as promoter engineering as a tool for specificity.
DNA delivery: AAV, Lentivirus, and Promoter Engineering
AAVs are the predominant vector platform employed by gene therapies in development. The widespread use of AAVs is due to their ability to mediate long-term gene expression in non-dividing cells, low risk of integration, lack of pathogenicity, and low immunogenicity compared to other viral vectors. This has led to an extensive body of preclinical and clinical expertise in AAV engineering developed over the past several decades.11 Nevertheless, targeted delivery remains a key challenge. Capsid engineering, which relies on modifying the viral capsid to achieve affinity for specific cell types, has several obstacles.
Modifying the AAV capsid to target specific cell types often compromises its ability to efficiently transduce cells, making it difficult to balance specificity and high infectivity.12 Extensive modifications can destabilize the capsid, impairing the packaging of the viral genome or preventing proper assembly and trafficking.13 Engineered capsids with novel surface topologies may also be recognized as foreign by the immune system, leading to neutralizing antibody responses and rapid clearance of the vector.14 In many cases, the approach to AAV capsid engineering is “blind” to the specific receptors or entry mechanisms used by the resulting capsid variants. The capsid, generated through iterative rounds of mutagenesis and selection, with desired tropism or transduction properties is selected based on functional readouts like transgene expression or viral genome accumulation in the target cells/tissues, without prior knowledge of the underlying receptor interactions.15 This can be challenging on its own, especially as (like with LNP targeting approaches) many pathogenic cells of interest are often similar on the cell surface, with which capsids interact, but crucially different inside the cell.
Lentiviral vectors offer several advantages, including a large packaging capacity and the ability to integrate into the host genome, enabling long-term transgene expression. However, achieving cell type specificity with lentiviral vectors remains a challenge.16 While lentiviruses can transduce a broad range of cell types, they often lack inherent tropism for specific target cells, such as stem cells or certain tissue types. To overcome this limitation, researchers have explored engineering the viral surface glycoproteins, which mediate cell entry. For instance, pseudotyping lentiviral vectors with glycoproteins from other viruses like Nipah virus or certain Rhabdoviruses has been investigated to alter their tropism.17,18 However, modifying surface glycoproteins can also impact viral titer and production yields, posing additional challenges for large-scale manufacturing.19 Furthermore, the integration of lentiviral vectors into the host genome carries a risk of insertional mutagenesis, which can potentially lead to secondary treatment-related oncogenesis.20 While this risk is lower compared to gamma-retroviral vectors, it remains a significant safety concern, particularly for in vivo applications.
Some therapies in development attempt to regulate gene expression at the level of transcription using engineered promoters that respond to specific transcription factors. This has the potential to mitigate potential toxicities associated with unregulated, long-term overexpression of a transgene. Even when a certain promoter is enriched in a cell type of interest (e.g., in cancer cells), it is not likely to be completely unique to that cell type. For example, the NF-KB, STAT and AP-1 families of transcription factors are known to be upregulated in both cancer and in inflammation.21 Additionally, for those few short synthetic promoters that are cell-type specific, their activity is often lower compared to ubiquitous promoters.22
This can result in suboptimal therapeutic levels of transgene expression.23 For lentiviruses, the integration site of the lentiviral vector within the host genome can influence the activity of the promoter due to local chromatin structure and regulatory elements. This position effect variability can lead to heterogeneous transgene expression levels among transduced cells.24 Additionally, AAV vectors have a limited cargo capacity of around 4.7-5 kb. However, many cell-type specific promoters, especially those driving robust expression and those capable of targeting diseased neurons, are large (often >10 kb) and leave little to no room for a therapeutic transgene. Attempts to shorten large promoters often result in significantly reduced promoter strength and transgene expression. Efforts to identify cell-type specific enhancers (e.g., through ATAC-seq) and combine them with minimal promoters often result in synthetic promoters that do not perform as expected in vivo due to a limited understanding of the interplay of regulatory elements required for robust, specific expression.25
RADAR: Leveraging the transcriptome as the source of specificity
By leveraging the transcriptome, Radar Therapeutics introduces precision-expressed mRNAs, a novel safety switch ensuring that a DNA- or mRNA-encoded drug is delivered only to diseased cells. This targeted approach allows for the selective removal or reprogramming of pathogenic cells, leveraging insights now published in Nature Biotechnology.26
Our approach begins with analyzing cells to identify disease markers or cell-state driver RNAs. We then design a highly specific "sensor" sequence containing a stop codon in front of the mRNA payload. In healthy cells without the marker RNAs, the payload is not expressed due to the stop codon, preventing translation, so that the mRNA defaults to an “off” state in all cells – overcoming a major limitation of the miRNA off-switches. However, in diseased cells with the marker RNA, the sensor and marker RNAs interact and thereby recruits an endogenous RNA-editing enzyme called ADAR. This interaction removes the stop codon, allowing the therapeutic protein payload to be expressed specifically in diseased or otherwise targeted cells. In contrast to leveraging ADAR and therapeutic guide RNAs to fix genetic mutations as is being done now in the clinic, RADAR uses ADAR to make a targeted fix to a synthetic therapeutic mRNA, using an endogenous RNA as the guide. Because the therapeutic mRNA is ours to design, including the “premature” stop codon that prevents expression in non-target cells, we can craft highly efficiently edited ADAR substrates within the therapeutic mRNA by leveraging decades of ADAR research as well as our own proprietary datasets.
This process is simple yet powerful. A technology like RADAR can interrogate most human genes, so that vast, multidimensional transcriptomic datasets can be mined to identify cellular signatures that distinguish cell types. These unique, distinguishing transcripts could be found in coding and non-coding RNAs, in intracellular, surface, or secreted markers. Unlike approaches that rely on luck (i.e., screening of large libraries of particles) to interrogate cell surface markers, our method uses rational design to specifically respond to disease and cell state drivers, allowing for highly targeted therapies.
Conclusion
It is likely that genetic medicines of the future will need to consider not only particle design, but payload design when considering specificity. Technologies like RADAR allow medicines precise enough in their expression to enable in vivo reprogrammability. RADAR allows for delivery efforts to focus on efficiency without getting hampered by the need for tight specificity, as the specificity challenge can be addressed at the payload expression level. We imagine in the future, all genetic medicines will be made with such tight regulatory control at the level of translation. When such precise genetic manipulation at the cell type and state level can be achieved, the future of DNA-based approaches and mRNA shines bright.
Sources
- Lin, Y., Cheng, Q. & Wei, T. Surface engineering of lipid nanoparticles: targeted nucleic acid delivery and beyond. Biophys. Rep. 9, 255–278 (2023).
- Kularatne, R. N., Crist, R. M. & Stern, S. T. The Future of Tissue-Targeted Lipid Nanoparticle-Mediated Nucleic Acid Delivery. Pharmaceuticals 15, 897 (2022).
- Lee, Y., Jeong, M., Park, J., Jung, H. & Lee, H. Immunogenicity of lipid nanoparticles and its impact on the efficacy of mRNA vaccines and therapeutics. Exp. Mol. Med. 55, 2085–2096 (2023).
- Lin, L., Su, K., Cheng, Q. & Liu, S. Targeting materials and strategies for RNA delivery. Theranostics 13, 4667–4693 (2023).
- Momin, M. Y., Gaddam, R. R., Kravitz, M., Gupta, A. & Vikram, A. The Challenges and Opportunities in the Development of MicroRNA Therapeutics: A Multidisciplinary Viewpoint. Cells 10, 3097 (2021).
- Zhang, S., Cheng, Z., Wang, Y. & Han, T.
The Risks of miRNA Therapeutics: In a Drug Target Perspective
. Drug Des. Devel. Ther. 15, 721–733 (2021). - Xie, Z., Wroblewska, L., Prochazka, L., Weiss, R. & Benenson, Y. Multi-input RNAi-based logic circuit for identification of specific cancer cells. Science 333, 1307–1311 (2011).
- Palomar-Siles, M. et al. Translational readthrough of nonsense mutant TP53 by mRNA incorporation of 5-Fluorouridine. Cell Death Dis. 13, 1–17 (2022).
- Floquet, C., Deforges, J., Rousset, J.-P. & Bidou, L. Rescue of non-sense mutated p53 tumor suppressor gene by aminoglycosides. Nucleic Acids Res. 39, 3350–3362 (2011).
- ERKUL, Y., YILMAZ, B. & OZDEMIR, C. Engineered oncoselective protein expression. (2022).
- Au, H. K. E., Isalan, M. & Mielcarek, M. Gene Therapy Advances: A Meta-Analysis of AAV Usage in Clinical Settings. Front. Med. 8, (2022).
- Nieuwenhuis, B. et al. Optimization of adeno-associated viral vector-mediated transduction of the corticospinal tract: comparison of four promoters. Gene Ther. 28, 56–74 (2021).
- Bohlen, M. O. et al. Adeno-Associated Virus Capsid-Promoter Interactions in the Brain Translate from Rat to the Nonhuman Primate. Hum. Gene Ther. 31, 1155–1168 (2020).
- Hamilton, B. A. & Wright, J. F. Challenges Posed by Immune Responses to AAV Vectors: Addressing Root Causes. Front. Immunol. 12, (2021).
- Lee, E. J., Guenther, C. M. & Suh, J. Adeno-Associated Virus (AAV) Vectors: Rational Design Strategies for Capsid Engineering. Curr. Opin. Biomed. Eng. 7, 58–63 (2018).
- Escors, D. & Breckpot, K. Lentiviral vectors in gene therapy: their current status and future potential. Arch. Immunol. Ther. Exp. (Warsz.) 58, 107–119 (2010).
- Maurice, M. et al. Efficient gene transfer into human primary blood lymphocytes by surface-engineered lentiviral vectors that display a T cell–activating polypeptide. Blood 99, 2342–2350 (2002).
- Humbel, M. et al. Maximizing lentiviral vector gene transfer in the CNS. Gene Ther. 28, 75–88 (2021).
- Natalia Elizalde, P. & Juan Carlos Ramírez, P. Lentiviral vectors: key challenges and new developments. Cell Gene Ther. Insights (2021) doi:10.18609/cgti.2021.002.
- Gene therapy clinical trial halted as cancer risk surfaces. https://www.science.org/content/article/gene-therapy-clinical-trial-halted-cancer-risk-surfaces.
- Transcription Factor AP 1 - an overview | ScienceDirect Topics. https://www.sciencedirect.com/topics/pharmacology-toxicology-and-pharmaceutical-science/transcription-factor-ap-1.
- Reddy, A. J. et al. Strategies for effectively modelling promoter-driven gene expression using transfer learning. bioRxiv 2023.02.24.529941 (2024) doi:10.1101/2023.02.24.529941.
- Rintz, E. et al. Promoter considerations in the design of lentiviral vectors for use in treating lysosomal storage diseases. Mol. Ther. Methods Clin. Dev. 24, 71–87 (2021).
- The Pros and Cons of Lentiviral and Adeno-Associated Viral Vectors. https://themedicinemaker.com/manufacture/the-pros-and-cons-of-lentiviral-and-adeno-associated-viral-vectors.
- Maturana, C. J. Engineered compact pan-neuronal promoter from Alphaherpesvirus LAP2 enhances target gene expression in the mouse brain and reduces tropism in the liver. Gene Ther. 31, 335–344 (2024).
- Kaseniit, K. E. et al. Modular, programmable RNA sensing using ADAR editing in living cells. Nat. Biotechnol. 41, 482–487 (2023).